Hemlock Overlook Regional Park: Proterozoic and Cambrian Metasediments

Figure 1. View looking upstream at the confluence of Bull Run and a minor tributary that is examined in this post (see Fig. 2 for location). The morphology of this area is typical for Northern Virginia’s Piedmont region; ridges and small plateaus dissected by many, irregular, often meandering streams, most of which flow into the Potomac River. Basement rocks are weathered on the ridges and are only visible along the streams which have deposited tens of feet of mixed sediment. The flood plain is several hundred yards wide in some locations, consisting of mud and silt; cobbles and some sand line the active channel, forming bars like those seen in this image.
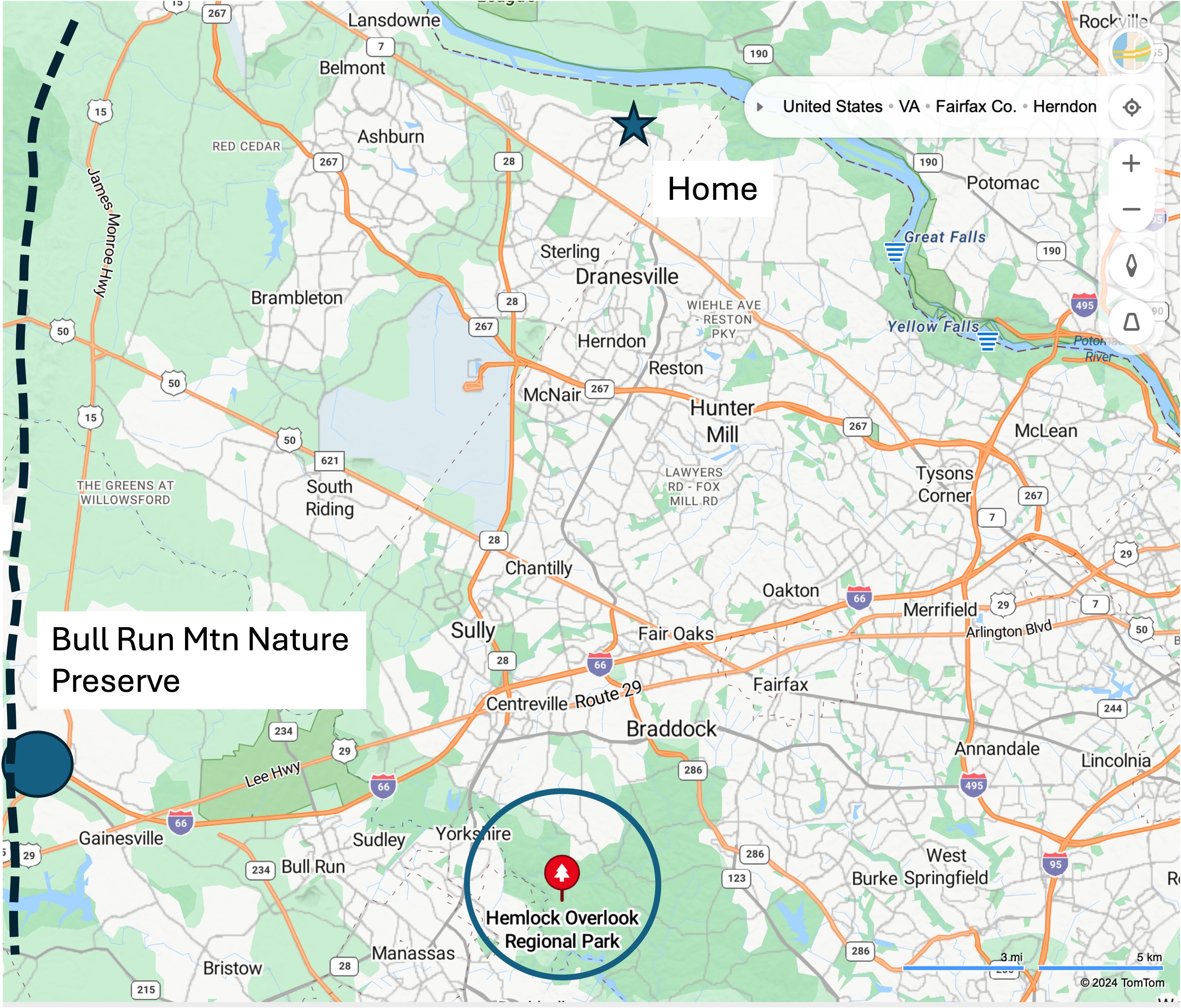
Figure 2. This map shows the area I have been exploring in recent posts. Hemlock Overlook Park is circled. Note that the study area is less than 10 miles from Bull Run Fault (dashed line), and an area we studied previously (indicated by the purple circle to the left of the map).

Figure 3. Photo of metasedimentary rocks exposed along the tributary creek shown in Fig. 3. These rocks have been rotated approximately 90 degrees and are vertical, with a northerly strike (not measured). They are fine grained and thin bedded, with thin layers weathering (fissile) in various locations within the study area.

Figure 4. Geologic map from Rock D of the study area. The blue dot is the location of Fig. 1. The green shade represents Cambrian metasediments (538-485 Ma). The lighter area is Proterozoic schist (1000 – 511 Ma). Our route followed Bull Run south (blue meandering line along right side of map).

Figure 5. Photo taken further to the south along Bull Run (see Fig. 4), where the rocks aren’t as weathered as further upstream. Their metamorphic grade is increasing as well.

Figure 6. When we entered the Neoproterozoic schist (light-shaded area ~800 m south of the blue dot in Fig. 4), the river channel narrowed, which allowed the construction of the first hydroelectric dam in the region. Note the vertical schist exposed on the other side of Bull Run.

Figure 7. Photo taken several hundred yards south of Fig. 6, showing the resistance of the schist to erosion. This rock hasn’t weathered much and appears to be a massive layer, but looks can be deceiving when dealing with rocks in the wild.
SUMMARY.
The rocks we saw today are typical for this region: a period of mud accumulation in deep water, burial many miles beneath the surface; the older (and more deeply buried) sediments were transformed into schist while the younger rocks were heated less under less pressure. The higher-grade metamorphic rocks (i.e. schist) are stronger and more resistant to weathering, creating a narrow canyon that allowed a dam to be constructed.
This was a continuous orogenic event that lasted from about one billion years ago to 500 million. Needless to say, there is more here than meets the eye, but the rocks are not library books …
Turkey Run State Park

Figure 1. View looking upstream in a small creek flowing into the Potomac River (see Fig. 2 for location). The hills are covered with a thin veneer of fine sediment deposited on Proterozoic and Paleozoic (i.e. 585-443 Ma) rocks. This image shows several ledges of this basement rock, which is the topic of this post.

Figure 2. Map showing Turkey Run Park relative to Washington DC. Further upstream, at Scotts Run (labeled on the map), we saw Proterozoic (2500 – 542 Ma) metamorphic rocks.
INTRUSIVE ROCKS

Figure 3. Outcrop of the Ordovician (488-423 Ma) tonalite, a medium-to-coarse-grained intrusive rock. Tonalite contains little or no quartz. This is a typical exposure in this area. This rock doesn’t form cliffs and the river isn’t contained within a narrow gorge as we saw further upstream. Large blocks have fallen away as the low and irregular bluffs erode. We’ll examine this tonalite in closer detail next.

Figure 4. Tonalite exposed further downstream from Fig. 1. Note the veins of light-colored minerals running through the rock. These are veins of quartz that filled fractures in the magma after it had cooled, but was still above quartz’s melting temperature. These veins are irregular because intrusive rocks aren’t layered like sedimentary rocks; they probably also reflect slow deformation of the semisolid magma on geologic time scales (i.e. millions of years).

Figure 5. Close-up of tonalite (image about 2 inches across), showing rectangular feldspar crystals and darker biotite and hornblende, which make up about half the composition. The darker minerals weather faster than the feldspar, leaving the latter protruding from the surface. This image also shows a slight foliation, running from the upper left to lower right. Foliation in igneous rocks can be syndepositional (i.e. as the magma cooled) or created when the solid rock is reheated enough to deform without breaking. I think these rocks fall in the first category.

Figure 6. This unusual image shows a layer of foliated tonalite sandwiched between two blocks that appear undeformed. This is probably an illusion caused by irregular weathering; however, Rock D reports this rock unit as containing fragments of older rock and previously solidified tonalite. The emplacement of a large batholith takes tens-of-millions of years to complete, during which time there was probably considerable crustal shortening associated with collisional plate tectonics. (Honestly, I wish I hadn’t taken this photo because it is really strange …)

Figure 7. Joint surface within the tonalite. Joints form when the magma has solidified and is brittle, tens if not hundreds of millions of years later. These joints were almost perfectly symmetrical, with 90 degrees between intersecting planes. Such an orientation suggests that the stress regime was uniform (horizontally and vertically). In other words, they occurred during uplift (isostatic stress regime) and not during an orogeny, i.e., they occurred a long time (geologically) after the events these rocks record.
METASEDIMENTARY ROCKS

Figure 8. This photo shows the approximate contact between the Ordovician intrusive tonalite and the older, overlying Cambrian (542-488 Ma) metasedimentary rocks (intrusive rocks come from deep within the earth). The boulders lying around are mixed lithologies, representing the two rocks. Whether the stream flowing towards the camera followed the contact (they are often weak points) or not is an open question.

Figure 9. Exposure of the Cambrian Sykesville Formation metasedimentary rocks into which the tonalite was intruded. These rocks were deposited in an ocean floor/deep-sea trench environment millions of years before they were buried deeply and heated enough to be metamorphosed. Their geologic age is uncertain but Rock D reports an age of 497-470 Ma. Considering that they had to be deposited and buried before being metamorphosed, it is reasonable to assume that they were deposited about 540 million-years ago. The tonalite age of 485-443 Ma is on firmer ground because this is an intrusive rock that can be dated by radioactive isotopes trapped in the minerals comprising it. Nevertheless, their ages overlap, which requires some explanation. Such large age ranges reflect the errors associated with dating rocks this old, but also the duration of orogenic events (I’ll get to that later). I tend to trust the oldest age reported because, when radiometric daughter products (used to calculate ages) escape from the rock, the apparent age will only decrease; thus I think the tonalite was intruded sporadically starting about 485 million-years ago. Note that the bedding is tilted to the right, which is to the west; I didn’t measure strike and dip, but the orientation is consistent with regional trends — dipping to the WNW at about 35 degrees.

Figure 10. Original bedding plane of the metasedimentary rocks. The lumpy surface is very similar to muddy sediments in modern submarine fan and trench settings, where sediment slides down steep slopes. Any fossils (if there ever were any) were destroyed during metamorphosis, which contributes to the dating problem I alluded to above.
FIELD RELATIONS
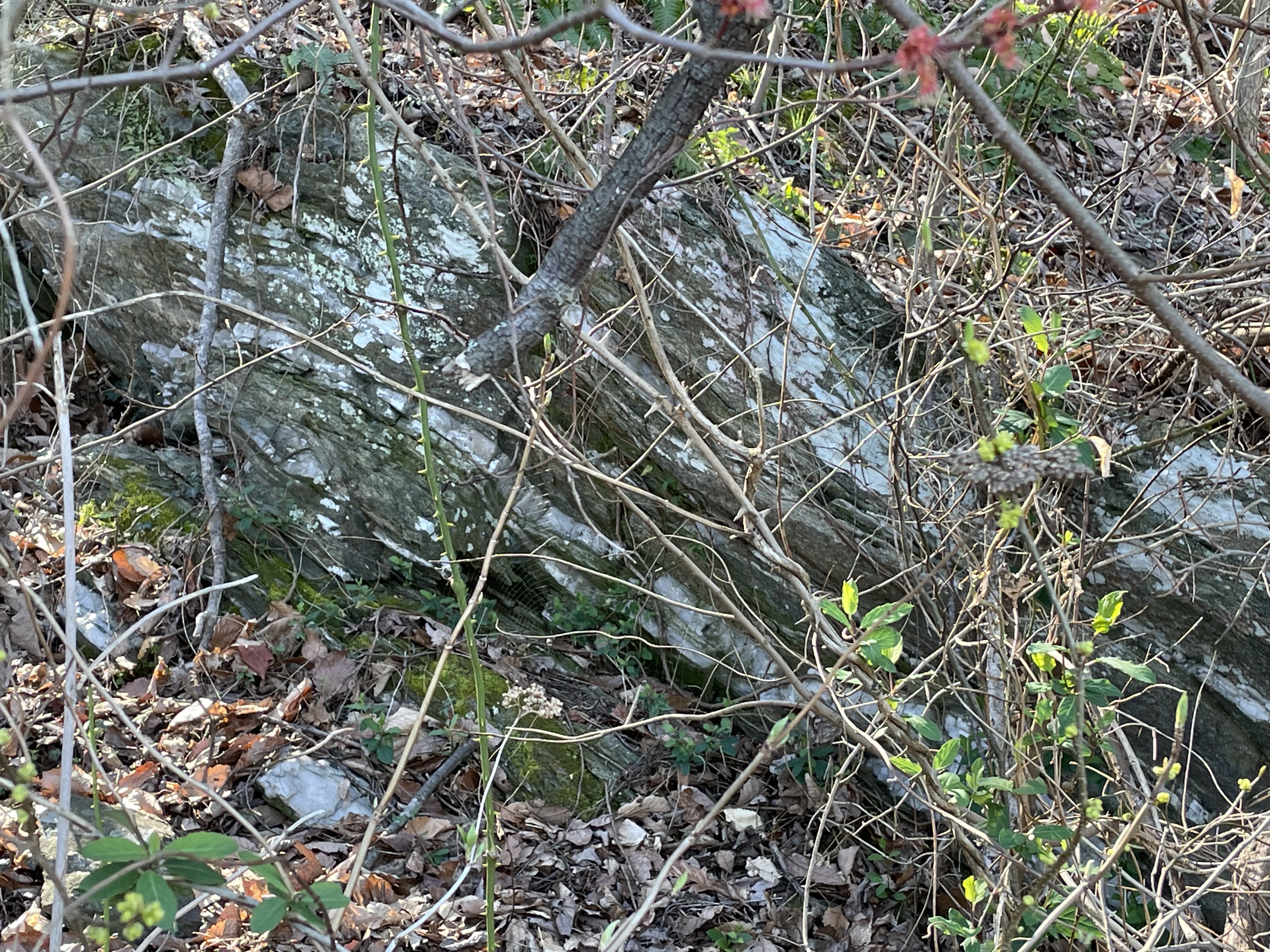
Figure 11. Exposure of the metasediments, showing light-colored layers within the darker beds of these rocks — exemplified by the thin layer just below the tree leaning to the right in the center of the photo (No, I didn’t tilt the camera; the tree is growing like that).

Figure 12. Photo of a block that fell away from the exposure seen in Fig. 11. This is mostly quartz but it contains several elongate fragments of metasedimentary rock. Although tonalite contains very little quartz, the original magma contained enough silica (Quartz is SiO4, pure silica) that magmatic fluids high in silica were infused into the overlying rocks as the magma cooled. Note that the country rock didn’t melt but was broken off during injection.

Figure 13. Exposure of a quartz-rich area within the metasedimentary rock.

Figure 14. Close-up of the exposure in Fig. 13, showing large quartz nodules. The red stains come from oxidation of iron-rich sediments in the country rock. The image is about two-feet across. The magma didn’t contain an abundance of rare-earth elements, but this looks like a pegmatite to me. Pegmatites form from excess quartz and other incompatible elements (e.g. lithium, tantalum, molybdenum) that remain in the last bit of semifluid as the magma cools; thus they are injected into the country rock as veins following weak zones (e.g. Fig. 11) to form large inclusions.
SUMMARY
We saw a lot today. More than 500 million-years ago, muddy sediments were deposited in a deep-sea environment, probably a subduction trench created as the Iapetus Ocean closed. This wasn’t a continental collision but more like we see in modern Japan or the Philippines. These sediments were buried over millions of years, finally heating enough under sufficient pressure to form new minerals but not obliterating their original sedimentary structures. About 485 million-years ago, magma rose from deep within the crust and forced its way into these ductile (but not molten) rocks, forcing high-pressure fluids rich in silicon along bedding planes, breaking off pieces of the older metasedimentary rocks and entraining them.
Today’s walk in the park took us to a time when an ocean basin was being subducted beneath a continent to form a supercontinent called Pangea.
These rocks record the Taconic Orogeny, the first in a series of collisional events that shook porto-north America to its roots. It was followed by continuous mountain building, culminating with the Allegehanian Orogeny, which ended approximately 260 million-years ago.
Radiometric ages tell us that the closing of Iapetus began at least 500 million-years ago and continued for 240 million years. However, the collision of tectonic plates isn’t defined by a single orogeny, as recorded in the rocks when continents are involved. I would like to point out that this massive tectonic event coincides with the evolution of animals, from the first mollusks to complex vertebrates like reptiles.
The evolution of life is closely correlated with plate tectonics so, the next time you see a rock in its natural state, take a moment to appreciate the debt we all owe to the earth …
New Year’s Day at Mather Gorge

Figure 1. View of Great Falls, Virginia, from the observation platform. If you look closely, you will see a contact between slightly lighter rocks to the left on the central island, and darker rocks to the right (the contact is just above the white water in the center of the photo). The lighter rocks are schist (originally mud) and the darker ones are metagraywacke (originally muddy sandstone with rock fragments) that were deposited continuously and metamorphosed about 1000 to 511 Ma. The image is looking north from the location shown by a star in Fig. 2. The muddy sandstone (i.e. metagraywacke) is older. We saw these rocks further downstream in a previous post. The transition from coarser to finer grained sediments suggests that an elevated region (e.g. mountains) was worn down over tens of millions of years.

Figure 2. Geologic map of Mather Gorge. The contact between the (originally) muddy sediment and coarser graywacke is shown by the dotted line. The star is located approximately. We examined the schist exposed at the observation area in a previous post. The area within the rectangle had many exposures of weathered metamorphic rock that was locally schist or metagraywacke. There is no unconformity in this area, so these rocks represent continuous deposition; thus, the metamorphosed sediments vary a lot between these two facies. We followed the river trail (white, dashed line closest to the Potomac River) south. The blue-gray line pointing NW from the Potomac is the old canal and not a modern stream, although there are several steep ravines leading to the river along Mather Gorge.

Figure 3. View looking upstream of a ravine leading to the Potomac. The waterfall is about 200 yards away and drops about 20 feet. The rocks are very thick bedded and steeply dipping to the WNW in this area, but their dip varies widely because of past tectonic deformation.

Figure 4. View looking downstream from the same location as in Fig. 3. The Potomac River can be seen in the upper center of the photo as a gray triangle pointing downward. Note the large blocks of metagraywacke clogging the channel and the steep face on the other side of the river. A nearby ravine like this was used to construct a series of locks to raise barges about 80 feet to enter an 18th century canal system (see Fig. 2 for location).

Figure 5. Bedding surface of metagraywacke, showing preserved bed forms suggestive of slumping. The raised surfaces are elongate ovals that indicate an original flow direction aligned with vertical in this photo’s orientation.

Figure 6. Close-up image (4x magnification) perpendicular to a bedding plane like that shown in Fig. 4. Thin bedding was deformed during burial by quartz inclusions (light colored, massive areas), which formed from silica squeezed out of clay layers containing a lot of water. The original muddy sediment was buried rapidly, trapping excess water within it. This sample doesn’t have sand or rock fragments visible at this magnification. The slightly golden area to the left of the center looked like pyrite (an iron sulfide mineral) but that’s just speculation; however, it is consistent with muddy sediment containing organic material.

Figure 7. This photograph reveals how plants speed up the weathering of rocks. The roots of this tree split the rock along weak planes, allowing water to penetrate and chemically breakdown the platy, micaceous minerals comprising schist.
The schists and metagraywackes we’ve seen exposed along more than ten miles of the Potomac River range from nearly horizontal to tilted almost vertical; in other words, this continuous sedimentary sequence is probably more than a mile thick. They were originally deposited in ocean trenches near a source such as volcanic islands (e.g. Japan), probably during subduction of ocean crust. Over tens of millions of years, volcanism ceased and the mountains eroded to produce massive volumes of mud. Thousands of feet of additional sediment (now eroded) would have buried these rocks deeply enough to recrystallize into more suitable mineral assemblages for the high pressure and temperature conditions of a subduction zone.
Before I speculate any further, I should address the steep and irregular angles at which we find these rocks today. This faulting is the result of extension within this area about 200 million years ago, during the breakup of a supercontinent called Pangea. Faulting is a brittle deformation process, which means that the rocks were not buried deeply at the time — a couple of miles, maybe.
Ignoring their steep angles, I haven’t seen evidence of folds in these rocks during my previous surveys in this area, which I find confusing, as an amateur field geologist. Radiometric dating suggests that they were metamorphosed between one billion and 500 million years ago; they were deposited tens of millions of years earlier so the radiometric age spans both deposition and metamorphosis. I don’t understand how sedimentary rocks were changed into metamorphic rocks in what was “obviously” a compressional tectonic regime (i.e. subduction of ocean crust), yet don’t show evidence of small-scale folding, a ductile process that occurs miles beneath the surface. Rocks of the Franciscan Complex (age 150 – 66 Ma) of Northern California, for example, are folded on scales of several feet; I guess these (unnamed) rocks of NOVA had such features erased during their (apparently) deeper burial, not to mention old age (1000 – 500 Ma) …
My confusing becomes vexing when I consider that these (now) metamorphic rocks weren’t deformed (i.e. folded) during the the plate-tectonic collision that created Pangea, a supercontinent assembled about 350 million-years ago. Geologists have long been disoriented by apparent contradictions like this, and they found a solution, which I alluded to in posts from Bull Run Nature Preserve and Shenandoah National Park.
This thick sequence of metasedimentary rocks must have formed part of an overthrust sheet, sliding between other layers of rocks, during the titanic collision that created Pangea. They were pushed far enough to the west that they weren’t assimilated by the Himalayan-scale mountain range that once ran the length of North America’s east coast. They were simply reburied by its sediments, but not deeply enough for their mineral composition to adjust; in other words, they had already seen the worst of their lifetime.
That’s my story … for now.
Proterozoic Metasediments at Scott’s Run

Figure 1. This post explored some of the Precambrian (1000 – 511 Ma) metamorphic rocks we’ve seen before along the Potomac River in Northern Virginia (NOVA). We followed Scott’s run to the waterfall (see Fig. 2 for location), where the creek dropped about ten feet at the current level of the Potomac. This photo shows a massive metasedimentary rock with no foliation. According to RockD this is a metagraywacke, which is a poorly sorted sand-silt sediment deposited on submarine fans (e.g. at the mouth of rivers), with distinctive structures that indicate slumping. The dates are indicative of the age of metamorphism rather than deposition. This is a thick formation but I couldn’t find a measured thickness but, based on its exposure along the Potomac, it must be thousands of feet thick; the large range in ages supports a model of continuous deposition/burial/low-grade metamorphism for hundreds of millions of years. Like a conveyor belt. There is no evidence of magmatism in this area, although a thick sequence of greenstone (metamorphosed basalt and associate volcaniclastic sediments) of this age is exposed in Shenandoah National Park.

Figure 2. Geologic map of Scott’s Run Nature Preserve. The waterfall (Fig. 1) is labeled in the inset map; the geologic map (right panel) indicates the exposure of metagraywacke (Pc Metaseds) in the area with thin lines. Our path followed Scott’s run to the left of the inset map, then the Potomac, before turning south at the Ridge, which will be discussed below.
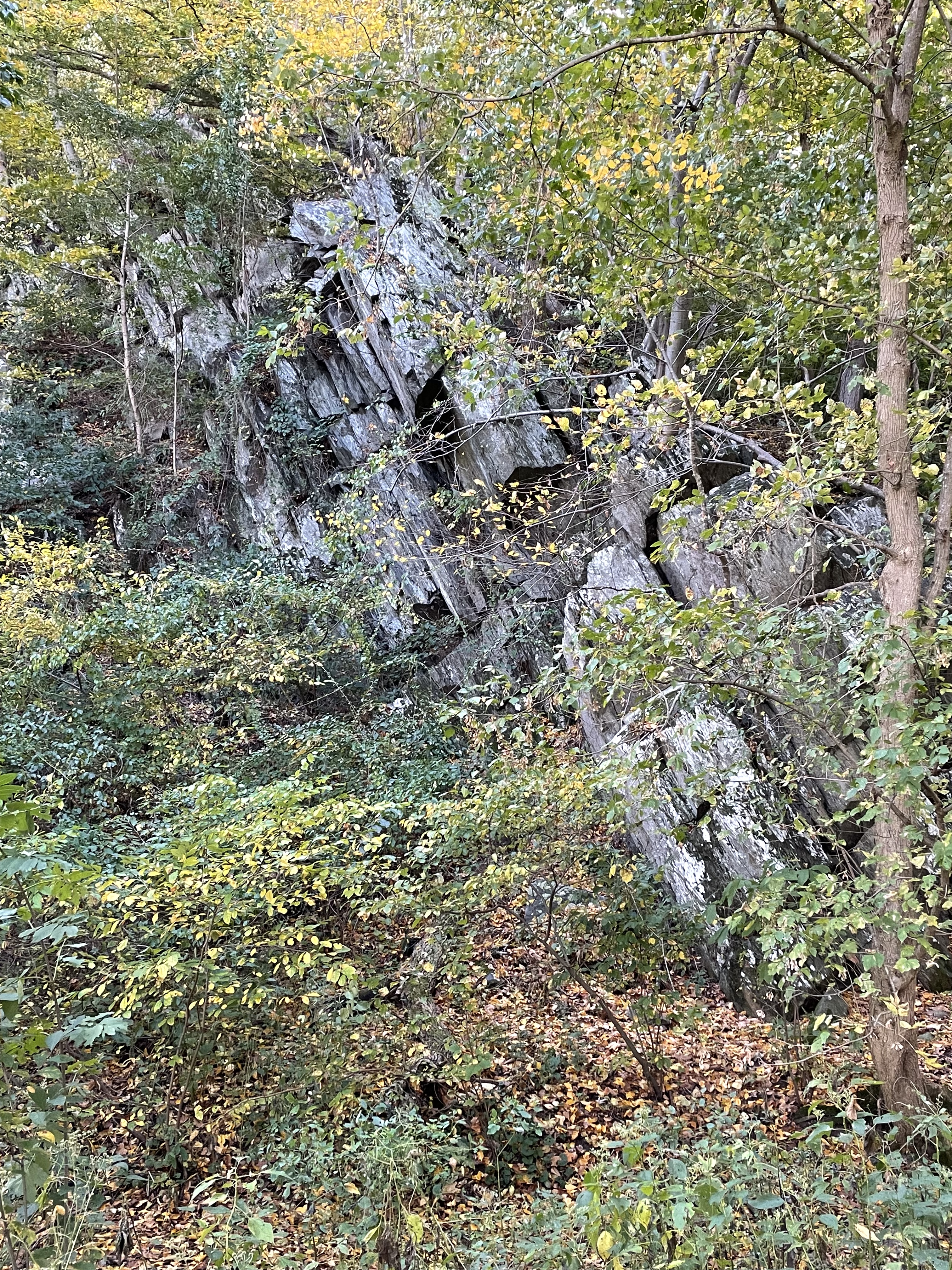
Figure 3. Photo of a ridge of schist (see Fig. 2 for location) the same age as the metagraywacke seen in Fig. 1. Schist is the result of burial and heating of mud at great pressure. Notice the distinct foliation of this outcrop, which is tilted about 70 degrees from horizontal to the west, the same trend we have seen throughout NOVA. The tilting is partly due to normal faulting during the rifting of Pangea about 200 million-years ago. I don’t know how these rocks’ original bedding (i.e. foliation) was deformed when they were buried and metamorphosed into schist. We’ll probably never know. The graywacke we saw in Fig. 1 has a large sand component, which tends to form thicker beds than mud; these schists were originally mud, with minor sand. The origin of foliation is complex; I conjecture that the high water content of mud (clay minerals have lots of bound water) leads to an excess of water that has no where to go when they are heated under pressure for long periods of time (i.e. millions of years). As new minerals crystallize, this water is forced into irregular layers (i.e. surfaces) within the sediments during diagenesis; I’ll go further and say (with significant justification)that sediments that slump into the deep-sea trenches that delineate subduction margins contain excess water and are rapidly buried. The result — schist is my answer.

Figure 4. Photo from the top of the cliff seen in Fig. 3, showing the foliation I was talking about. This ridge (see Fig. 2 for location) is demarcated by short streams with steep slopes on both sides. Here, about 60 feet above the Potomac, it narrows to less than 10 feet along its spine.

Figure 5. This image reflects the incredible power of water on a metamorphic rock filled with excess water. Mud doesn’t form quartz or feldspar (chemically resistant minerals) during low-to-medium-grade metamorphism because there isn’t enough silicon in these minerals; they consist of layers of aluminum, iron, calcium, etc, bound with silicon and sandwiched between what are basically water molecules. The result is a fissile rock like this, where the slivers of resistant minerals are getting worn away.

Figure 6. This photo, taken less than 20 feet from Fig. 5, shows the end result of the microscopic weathering of schist. It is turning into mud as the trapped water is released and the aluminosilicates (clays) break down into smaller and smaller grains. This soil is rich in aluminum and is mined for that element in tropical areas where plentiful rainfall maintains the conveyer belt that recycles mud into mud.
Summary. Between one-billion and five-hundred-million years ago, NOVA was a deep sea trench, probably associate with subduction of oceanic lithosphere beneath another tectonic plate. There were, doubtless, interludes in this process, but the geologic evidence suggests that subduction was the game plan. Muddy sediments eroded from what were probably volcanic islands (i.e. the Catoctin Formation) and slid down deep slopes into trenches like we see today in the western Pacific.
This process was heterogenous just as in the modern world. Volcanic activity was dominant in Shenandoah National Park, less than a hundred miles west of Scott’s Run Nature Preserve. The best image I can conjure up is the Philippine Islands, which the irresistible force of mantle convection is crushing against the immovable object of the Eurasian tectonic plate.
I am in awe of the planet we inhabit …
Shenandoah National Forest: Precambrian Volcanism

Figure 1. View looking SW at Shenandoah Valley from Miller’s Head (3484 feet elevation). The forested hills to the center-left are Precambrian metamorphic rocks (1600 – 1000 Ma), separated by an arcuate fault from Paleozoic sedimentary rocks (540 – 250 Ma) that get younger to the west. The ridge in the distance is constructed of Devonian rocks (416 – 360 Ma) whereas the closer ridge is Ordovician to Silurian sandstone (443 – 419 Ma). Today’s post will examine the Precambrian rocks that comprise the Blue Ridge Mountains, which we saw in a the last post and in a previous post.

Figure 2. (A) Map of northern Virginia (NOVA) showing my home (star), Washington DC, and the study area in Shenandoah National Park (rectangle in lower left). The inset photos show the stunning views to be found in the lower part of the study area. (B) Geologic map of the study area, showing Precambrian rocks in pink shades and Cambrian rocks in brown, Ordovician in green, and Devonian strata in orange shades. The Shenandoah Valley forms a syncline with smaller folds contained within it, like folds in a rug. Younger rocks are exposed by erosion along the axis of a syncline. The solid lines are faults that have been identified in the field although the kind of fault can’t always be determined.

Figure 3. Images from Hawksbill Mountain (elevation 4042 feet), the highest point in Shenandoah National Park. (A) View looking north, showing bedding planes dipping to the SE as we’ve seen throughout NOVA. This is the general structural trend along the eastern margin of North America. These volcaniclastic deposits are part of the Catoctin Formation, dated by radioisotopes to between 1000 and 485 Ma. (B) A close-up shows that these rocks are fissile, which means they are forming thin layers as they weather. They were originally very fine grained, possibly ash or mud (clay minerals) and other weathering products. (C) This photo reveals (despite the dappled shade of trees) a contact (yellow line) between tan and bluish rock that has no other distinguishing features. This must be caused by a slight difference in composition and/or texture that leads to subtle variations in reflected light; if I may speculate, I think the blue ash/sediment filled a depression in the tan material; however, this conjecture is based on the physical environment when this volcanic material was produced. Eruptions were not continuous and there was always a slightly weathered surface upon which new ash was deposited. (D) Outcrop of Catoctin Formation rocks about 600 feet lower than the summit, and much further down-section from the rocks seen in plate A. This basalt/ash was deposited millions of years before what we see in plate A and the magma chamber would have evolved substantially. The original bedding is, as near as I could tell, nearly horizontal rather than tilted to the SE. This implies that brittle deformation (i.e. faulting), which occurred tens of millions of years after eruption, subsequent burial, and metamorphosis, was localized within the larger body of Catoctin Formation rocks (3000 feet thick and extending for many miles); in other words, these metabasalts were hard as rocks (as they say) when they were compressed horizontally. Perhaps they were even transported tens of miles along a thrust fault? We saw evidence of a Precambrian thrust fault at Bull Run Nature Preserve.

Figure 4. Catoctin Formation metabasalts at Dark Hollow Falls. (A) These rocks don’t form sheer cliffs, so this creek flows over a series of ledges for a vertical distance of about 70 feet.(B) The bedding is tilting to the SE as at Hawksbill summit and contains fissile layers as seen here, intercalated with massive units as seen in plate A. Also note the dark layer in the center of the photo, which may be similar in origin to the blue layer seen in Fig. 3C. (C) Representative boulder of the rocks at Hollow Falls. Notice the white flecks in this sample, which is about 18 inches in length. (D) Close-up of the larger light-colored ellipse in the lower-left part of plate C, showing concentric rings of light material (probably quartz) in a fine matrix (probably basalt). This is an amygdule, which is a mineral filling a cavity in a volcanic rock that is filled with vesicles. The vesicles are originally pockets of volcanic gasses that are trapped when the basalt is erupted, and then fill with hydrothermal fluids which deposit minerals. (E) Bedding surface showing many semicircular ridges, which are (probably) remnant from when this basalt was exposed to the atmosphere and the gases escaped; in other words, this was the top of a basalt flow whereas plate D was too deep within the flow for the gas to escape before the basalt solidified. A moment in time frozen for more than 500 million years.

Figure 5. Photos of Miller’s Head. (A) View looking west (see Fig. 2B for the geologic map). The yellow lines approximately outline faults within the Paleozoic rocks underlying Shenandoah Valley; note that one curves to the west where Neoproterozoic (1000 – 542 Ma) rocks jut into the valley. The second fault borders a low ridge comprised of the same rocks we found on this peak. (B) Vertical joint that shows intersecting joints (the X’s seen in the rock face). Water seeps in through this system of fractures and weathers the rock into blocks. (C) The result of this weathering process is a mountain covered by a veneer of rubble. The entire mountain in this area is turning into a pile of boulders that look like they were pushed aside by a bulldozer, but they haven’t moved other than sliding over one another down the steep slope. (D) Close-up (4X magnification) of a fresh surface of this rock, which is Charnockite, a granitoid rock that contains pyroxene minerals, which do not occur in granite. This sample reveals quartz (Q), plagioclase feldspar (Pf), potassium feldspar (Kf), and a lot of pyroxene (Px). Note that Pf is white and not dark, which would be more indicative of a mantle source, and pink Kf which is typical for a granite formed from crustal material. Charnockites are enigmatic and almost entirely found in Precambrian rocks. According to RockD (radiometric dating), these intrusive rocks are between 1600 and 1000 million-years old.
Summary. This post doesn’t add anything new to what we’ve already learned from previous field trips but it reinforces the picture that has been developing from our previous posts in NOVA and elsewhere; tectonic plates were colliding along the eastern margin of North America as long ago as 1.6 billion years, while muddy sediments were being deposited in deep water (below wave base or rivers), and continued doing so until about 500 million-years ago. This was a discontinuous process and, considering the billion years duration of this tectonic upheaval, it is possible that multiple mantle plumes were competing for space. Such a huge span of time could easily encompass more than one Wilson Cycle, but the best I can say in this post is that the Proterozoic (2500 – 542 Ma) looks to have been as active an age as we’ve seen in the last 500 million years.
One final note. The earth is cooling very slowly but, nevertheless, it was hotter in the Precambrian. This means that plate tectonics, driven by mantle upwelling (i.e. plumes) would have been more vigorous although not by an order of magnitude. Thus, given the immense span of time between the Middle Proterozoic (1600 – 1000 Ma) Charnockite we encountered (Fig. 5) on this trip and the Catoctin Metabasalts (1000 – 485 Ma), it is safe to say that we haven’t seen the whole story.
The rocks speak softly but they know the truth …
Shenandoah National Park: Precambrian Volcaniclastic Rocks

Figure 1. View looking west from Dickey Ridge/Hill (See Fig. 2) towards the Shenandoah Valley. This is where the Ridge and Valley province begins, a series of elongate mountains running approximately 30 degrees east of north, the same as the structural trend we’ve seen in previous locations throughout northern Virginia. Dickey Hill has an elevation of 2427 feet, about 1800 feet higher than the valley floor.

Figure 2. The left image is a map of Shenandoah National Park, which extends along the ridge line of the Blue Ridge Mountains. The study area is circled and a geologic map from RockD is shown in the right image. The blue dot is where we started our climb to Dickey Hill. The rocks of this area (shown in a light-gray color) are the Catoctin Formation (1000 – 485 Ma): metabasalt (metamorphosed to greenschist facies), including some preserved volcanic structures, which are the subject of this post.

Figure 3. Exposure of Catoctin metabasalts, showing original bedding, which is dipping away from the camera, and variability of these rocks in outcrop.

Figure 4. Example of what metabasalts look like in the field. (A) boulder showing a greenish hue that has been roughed up a bit and is no longer angular. (B) Close up of area enclosed in (A), showing some of the biological materials that produce the mottled appearance of these rocks. The bright areas with angular form are probably quartz and feldspar, two minerals commonly associated with greenschist facies metamorphism (low pressure and low temperature).

Figure 5. Example of textures found in Catoctin metabasalts: (A) bluish-green boulder with angular form. (B) Close up of fine-scale textures preserved from the original basalt. The acicular sections result from a preferred alignment of minerals, indicating a flow direction; blocky texture indicates that this part of the sample was part of a larger flow and probably more viscous; nodular textures probably result from weathering at some point, possibly soon after deposition. Volcanic flows are not uniform; for example, the basalts of the Catoctin formation are 3000 feet thick but were extruded over tens of millions of years, during which the magma chamber would have changed in composition. These “nodules” are elongate and could be due to recent (last few million years) weathering of acicular textures. Perhaps nodular is the wrong word; these also look a lot like miniature pahoehoe lava, which is ropy when first created.

Figure 6. (A) angular boulder with a rich blue color, suggestive of blueschist facies metamorphism (low temperature and high pressure). (B) Close-up showing ropy, flowing structures around fragments of basalt (originally) that were carried with the flow. We saw this kind of flow structure at Koko Crater on Oahu in a previous post, but those rocks were only a few million years old and hadn’t been buried and subjected to huge tectonic stresses.

Figure 7. Images of volcanic textures in an outcrops. (A) This photo shows a slight difference in texture between the intrusion and the surrounding lava, even though both were erupted at the same time. The lava produced by a magma chamber, especially near the surface, is not homogeneous but rather a poorly mixed assortment of molten and solid material infused with high-pressure volcanic gas. The circled area labeled as a Cavity (speculative) is an example of this heterogeneous volcanic texture. (B) This photo of an outcrop shows angular fragments quite distinct from the background matrix (not to be confused with lichen); these are not original textures because basalt does not contain light-colored rock fragments. These inclusions are metamorphic in origin, probably quartz and feldspar.

Figure 8. This hand sample was photographed at the top of Dickey Hill. It is as fresh a sample as you can get without a rock-hammer. Note the thin filament of material separating two conchoidal fracture zones. The greenish color is why metabasalts are called greenschist.

Figure 9. This photo shows a couple of post-tectonic textures that reflect events after these volcanic rocks were buried and metamorphosed. Joints are brittle fractures that occur when a rock has been exhumed and the stress regime has reduced; the rocks break in patterns like the “X” that has been superimposed on this image. Joints cannot be dated so all I can say is that this pattern, which was expressed in all of the outcrops I saw, occurred millions of years after burial, probably after the break-up of Pangea, when the mountains that once overlay this area were eroded away. The circled area shows rounded corners like we saw in Fig. 5B, suggesting that water flowed over this outcrop for a long period of time. The fracturing in Fig. 8 would have occurred during this period of relaxing stress.
SUMMARY. About one-billion years ago, lava flowed onto the land that later became Virginia for millions of years, culminating in a 3000-foot-thick pile of basalt. This is half as thick as the Deccan Traps basalt province in India or the Columbia Plateau of North America; the latter was produced in 10-15 million years. Both of these geologic provinces are associated with collisional tectonic regimes.
The uncertainty in age for the Catoctin Formation (1000-485 Ma) is due to the uncertainties of radiometric dating, caused mostly by loss of radioactive products over time (loss of products gives false young ages); thus, it is probably safe to say that these rocks were originally produced about one-billion years ago.
We saw these rocks at Morven Park and Catoctin Creek, and we saw contemporaneous sedimentary rocks at Bull Run nature preserve. If these basalts are analogous (that’s a big IF) to the Deccan Traps and the Columbia Plateau, this was a collision of tectonic plates. This tentative interpretation is supported by the lack of pillow lava structures (lava erupted into deep water) reported in the Catoctin Formation. The presence of terrigenous sedimentary rocks deposited 500 million years later in Virginia suggests that an entire Wilson Cycle (opening and closing of an oceianbasin) occurred between the Catoctin formation (~1000 Ma) and the Harpers formation (~538 Ma).
The greenschist metabasalts of the Catoctin formation weren’t buried deeply or heated very much, so they weren’t close to the point of impact during the closing of Iapetus Ocean, which was somewhere on the continental shelf a hundred miles or more east of the study area. This tectonic collision began north of Virginia about 550 million-years ago (i.e. when the Harpers formation was deposited). It is called the Taconic Orogeny. The result was the supercontinent Pangea, which lasted several-hundred million years before being torn apart about 200 million-years ago.
Tectonic plates are dancing but who’s playing the music?
Mantle plumes are elusive for humans to track. Imagine a pan of boiling water; bubbles appear, some large, some small, spaced at seemingly random distances apart. They last only as long as it takes them to rise to the surface.
The rocks are trying to tell us what dance they are moving to …
Catoctin Creek: Deep into the Precambrian

Figure 1. The Waterford mill was constructed in the 1820’s and operated until 1939. It was located along Catoctin Creek because there is a significant drop in stream elevation here. A low ridge behind the mill suggests part of the reason: resistant rocks that produce a series of sudden drops in the stream, perfect for collecting water behind a dam not too far from the mill. The shorter the length of the “head race”, the lower the construction costs. The “tail race” can be seen leading from the waterwheel. This small ditch leads around the facility and drains into Catoctin Creek a couple hundred yards downstream. This post will explore upstream on Catoctin Creek, as far as the mill pond (storage pond for hydraulic pressure), and see what the rocks tell us.

Figure 2. (A) Regional map of the study area. My house is labeled with a star. Bull Run fault is a continuous normal fault that we saw at Morven Park and Banshee Reeks Nature Preserve; its location in the figure is approximate. It demarcates younger Mesozoic rocks to the east from older, Precambrian, rocks to the west; the latter form an elongate ridge that cuts through the study area (rectangle centered on Waterford), as well as the Blue Ridge further west, which front the Shenandoah Valley. Vertical movement along Bull Run fault began about 220 Ma (Ma is a million years, but measured by radiometric techniques) when Pangea was torn apart by the newly forming Atlantic Ocean. (B) Geologic map from RockD of Waterford area. The section of Catoctin Creek discussed in this post is enclosed by a blue ellipse. Note that the Precambrian rocks fall into two distinct sequences: 1.6 Ga (billion years measured with radioisotopes) and gneiss, both metamorphosed; and 1 Ga to 600 Ma volcanics and associated sedimentary rocks. The contact between them is an unconformity but the type cannot be determined from field data. This might be a buried and disrupted late Precambrian (~600 my) thrust fault that pushed older rocks over younger, like we saw at Bull Run Nature Preserve.

Figure 3. (A) View looking downstream along Catoctin Creek, showing rounded cobbles (~2 inches) in a sandy matrix, with silt and mud. This unlikely assortment of sediment grain sizes suggests to me that there are multiple sources being mixed along the creek; for example, rounded cobbles suggest miles of transport along swift-flowing creeks whereas mud is the product of physical and chemical weathering of rocks with a small quartz content (quartz is very hard and chemically stable; i.e. sandy beaches). (B) Exposure of older Precambrian schist along the creek bed, forming a low obstruction. (C) The schist layers (schist is a fissile rock) present a weathered appearance; chemical weathering produces mud in-situ without bedload transport, producing few cobbles.

Figure 4. Examples of different rock types found along Catoctin Creek. (A) Collection of angular schist boulders (~one foot in size) at one of the tributaries to the main stream. (B) Quartz intrusion, probably from the oldest rocks (metamorphosed granites and gneisses). The sample is about one foot long. (C) Fresh surface of the schist, showing fissility and a sheen associated with lower-grade metamorphosis, such as in phyllite. This sample was several feet long and had been transported to the mill-pond dam during construction of the mill.

Figure 5. The dam constructed to retain water for Waterford mill contains a variety of large boulders, but the majority were schist (see Fig. 4C). I didn’t see any granite or gneiss, which isn’t surprising because these large stones would have been difficult to transport in the early nineteenth century. They used what was readily available; the exposure of schist along the creek bed (Fig. 3A) suggests that the ridge fronting the mill (Fig. 1) was a likely source of material; after all, rock had to be removed to build the mill and the town of Waterford.
SUMMARY.
More than 1.5 billion years ago, something was happening in Loudon County, Virginia, long before there were multicellular organisms (eukaryotes) or even land plants. There was a collision of tectonic plates massive enough to produce granite and gneiss (high-grade metamorphism) and then deform these very durable rocks.
Four-hundred-million years later, an episode of extreme volcanism occurred and thick sequences of basalt and volcaniclastic sediments were laid down in Loudon County at about the same time (give or take a hundred million years) as deeply buried shales were being transformed into schist at several locations along the eastern margin of modern North America: less than 50 miles from Waterford, at Great Falls; what would become New York City; and Vermont. This was the closing of Iapetus, which took hundreds of millions of years and stretched for thousands of miles, creating Pangea and the rise of eukaryotes, fish, amphibians, reptiles, and mammals, not to mention land plants.
About two-hundred-million years ago, Pangea was torn apart and grabens formed, filling with sediment eroded from the surrounding elevated terrain. These sedimentary rocks are found east of Bull Run fault (Fig. 2A) where they remained protected from the elements (chemical and physical weathering) while the older rocks were elevated to form mountain peaks in the modern world and eroded into boulders, sand, and mud.
Two-hundred-million years later, the shattered remnants of a once-majestic mountain range, stretching from Canada to the Gulf of Mexico, comprise its core of metamorphic and igneous rocks recording events we can only speculate about today.
From There to Here: Wyoming

We discussed the tumultuous history of Precambrian rocks in Montana in my last post. The story of crustal shortening in western North America continues to this day. The huge, shield volcanoes comprising the Cascades Mountains show that this westward motion has not ceased at the current time.
The story of oceanic subduction and collision with multiple microcontinents is recorded in the rocks I had to drive past, so I have to resort to a geological map again.

Summary. This was a short post because I was occupied and didn’t have time to explore this fascinating region. Nevertheless, I can confidently say that when Pangea broke up, the North American tectonic plate began to “swallow” the proto-Pacific plate and any microcontinents it harbored.
This 200 my long process created the Rocky Mountains, the overthrust belts of Montana, the Black Hills of South Dakota, the Colorado Plateau, the volcanic Cascade Mountains, the complex system of faults that define California, and so many other geological features of the western North American craton.
It wasn’t as if another gigantic mountain range could form in the aftermath of Pangea’s break-up. The earth can only produce one of them every couple hundred million years, a tectonic pattern called a Wilson Cycle.
Spokane, Washington, to Gillette, Wyoming: Geology in the Rearview Mirror
This post is experimental and not particularly interesting, but it is the best I can do under the circumstances; I followed Interstate 90 through the Rocky Mountains at 70 mph, with no pull-offs, and trying to take photos in the heavy traffic would have been suicidal. Instead of including a map, photos of outcrops, and some close-ups to examine mineralogy, I am relying on geological maps and my memory. The most experimental part is that I’m working on an iPad, which is a blessing and a curse. Let’s see how it worked out.





Summary. The oldest rocks (Precambrian metasediments shown in purple shades) are scattered throughout the Rocky Mountains. These old rocks were pushed and pulled for hundreds of millions of years as microcontinents collided to form what we call western North America.
Paleozoic rocks (500-230 my) that would have been deposited on top of them, or intruded into them, are only found in scraps here and there (I’m speculating, but Paleozoic rocks have a habit of turning up in the unlikeliest places).
During the late Cretaceous (about 80 my), granitoid intrusions forced their way into these older rocks, as I saw at Butte and other small mountain ranges (pink and tan hues). This was a geologically active period in the evolution of western North America.
About fifty-million years ago, volcanoes formed along the western margin of North America (e.g. Mt Hood and other volcanoes produced thousands of feet of volcanic rock, forming the Columbia plateau (yellow shades in Fig. 5). At approximately the same time (50 – 5 my) sediment was collecting in lakes and shallow inland seas leftover from the Cretaceous Interior Seaway. These sediments are undeformed and not very well lithified (i.e. not buried deeply); they appear east of Bozeman MT as green in Fig. 5.
Hidden beneath the Precambrian rocks, which were pushed eastward as much as 150 miles in Canada, and Miocene sediments, lay the oil and coal rich Cretaceous sediments laid down between about 150 and 60 my ago. As proof of this, Billings MT (rightmost circled area in Fig. 2), with a population less than 150 thousand, has three oil refineries; but it is so remote, with so little infrastructure (e.g. pipelines), that trucks deliver refined petroleum products to rail cars. It is a modern western boom town.
We’ll see what tomorrow brings …
A question of scale: Indian Canyon Falls




Summary. I found Indian Canyon falls by looking for Lake Missoula park, which turned out to be closed to the public. However, the Park Service supplied a link to other geological attractions, with navigation instructions—GoogleMap took me to a nondescript, heavily wooded area, where I found something I hadn’t expected to see.
The rivulet of water flowing over the “fall” during the dry season is an omen of what is to come for this relatively unknown canyon (it was actually covered with biking and hiking trails). At first the trickle carries only mud, then sand, then gravel, then boulders, then …
Indian Canyon falls is how it begins. Where it ends …
Think big …
Recent Comments